1x2 Step-Index Multimode Fiber Optic Couplers, Ø200 µm Core, 0.39 NA
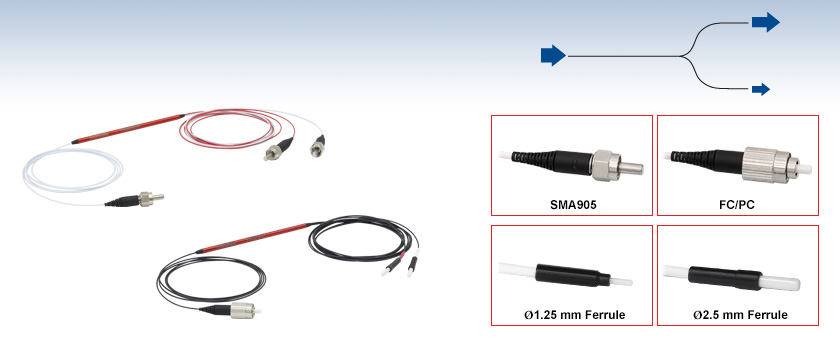
- Multimode Couplers with Ø200 µm Core, 0.39 NA Step-Index Fiber
- 400 - 900 nm or 400 - 2200 nm Wavelength Range
- Inputs with FC/PC or SMA905 Connectors
- Versions Available with Ø1.25 mm or Ø2.5 mm Ferrules Terminating Both Outputs
Use for
Splitting Signals
TT200R2S1A
90:10 Coupler with
SMA905 Connectors
TT200FS1A
50:50 Coupler with
FC/PC Connector
and Ø2.5 mm Ferrules

Please Wait
1x2 MM Coupler Selection Guide |
---|
Graded-Index Fiber |
Ø50 µm Core, 0.20 NA, 850 nm & 1310 nm |
Ø62.5 µm Core, 0.275 NA, 850 nm & 1310 nm |
Step-Index Fiber |
Ø50 µm Core, 0.22 NA, 400 - 2200 nm (High and Low OH) |
Ø105 µm Core, 0.22 NA, 400 - 2200 nm (High and Low OH) |
Ø200 µm Core, 0.22 NA, 400 - 2200 nm (High and Low OH) |
Ø200 µm Core, 0.39 NA, 400 - 2200 nm (High and Low OH) |
Ø200 µm Core, 0.50 NA, 400 - 2200 nm (High and Low OH) |
Ø300 µm Core, 0.39 NA, 400 - 2200 nm (Low OH) |
Ø400 µm Core, 0.22 NA, 400 - 2200 nm (Low OH) |
Ø400 µm Core, 0.39 NA, 400 - 2200 nm (Low OH) |
Ø400 µm Core, 0.50 NA, 400 - 2200 nm (Low OH) |
Ø600 µm Core, 0.39 NA, 400 - 2200 nm (Low OH) |

Click for Details
Each coupler is engraved with the item #, serial number, and key specifications for easy identification. In the coupler shown above, when the white port on the left is used as the input, the coupling ratios listed below correspond to the ratio of the measured output power from the white (signal output) port to the red (tap output) port.

Features
- Ø200 µm Core Multimode Couplers
- 50:50, 75:25, or 90:10 Split Ratio
- 400 - 900 nm (High OH) or 400 - 2200 nm (Low OH) Wavelength Range
- Max Power Level (See Damage Threshold Tab for Details)
- 5 W (With Connectors or Bare Fiber)
- 10 W (Spliced)
- SMA905 or 2.0 mm Narrow Key FC/PC Connectors
- 50:50 Couplers Available with Ø1.25 mm or Ø2.5 mm Ceramic Ferrules on
Output Ports - 0.8 m Long Fiber Leads with a Tolerance of +0.075 m / -0.0 m
- Individual Test Reports Available for Each Coupler; Click Here for a Sample
Test Report - Contact Us for Custom Coupling Ratio and Connector Options
Thorlabs' 1x2 Multimode Fiber Optic Couplers are designed to split light over a 400 - 900 nm (high OH) or a 400 - 2200 nm (low OH) wavelength range. High-OH fiber is preferred for the visible region, while low-OH fiber is preferred for the infrared region and telecom applications. Couplers with a Ø200 µm core fiber and a 0.39 NA are featured on this page and are available with 50:50, 75:25, or 90:10 coupling ratios. These couplers have a max power level of 5 W with connectors or unterminated (bare) fiber, or 10 W when spliced (see the Damage Threshold tab for more details). For best performance, these step-index couplers should be used with an incoherent or multimode light source as described in the Launch Conditions tab.
1x2 couplers are manufactured using the same process as our 2x2 fiber optic couplers, but have only one input port for simplified use and cable management. These couplers are ideal for applications where light is split from the input port into two output ports at the specified coupling ratio and are not recommended for use as combiners due to power losses; see our selection of 3x1 Multimode Combiners for combining applications. The unused port is internally terminated within the coupler housing in a manner that minimizes back reflections (please see the 1x2 Coupler Tutorial tab for details).
Thorlabs provides an individual test report for each coupler that contains measured test data verifying performance. A sample test report for our Ø200 µm core multimode, 0.39 NA couplers can be viewed here.
These couplers are offered from stock with SMA905 or 2.0 mm narrow key FC/PC connectors. We also offer 50:50 couplers with SMA905 or FC/PC connectors on the input port and either Ø1.25 mm or Ø2.5 mm ceramic ferrules on the output ports.
Custom and OEM Fiber Couplers
Our couplers are produced on-site in our North American manufacturing facilities and our design team is able to deliver custom solutions in as little as three weeks. Custom coupler configurations with other wavelengths, fiber types, coupling ratios, port configurations, connectors, or housing options are available, and each custom coupler includes an individualized test report. Please contact Tech Sales for inquiries or to discuss your application.
Thorlabs also offers Ø200 µm core fiber couplers in a 2x2 configuration; they can be found here.
Alternative Fiber Coupler & Splitter Options | ||||||||||
---|---|---|---|---|---|---|---|---|---|---|
Double-Clad Couplers | Single Mode Couplers | Single Mode Planar-Lightwave-Circuit Splitters |
Multimode Couplers | Polarization-Maintaining Couplers | Wavelength Division Multiplexers (WDM) |
|||||
2x2 | 1x2 | 2x2 | 1x4 | 1x8 | 1x16 | 1x2 | 2x2 | 1x2 | 2x2 |
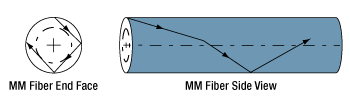
Click to Enlarge
Figure 63A Diagram of Skew Ray Propagation
Step-Index Multimode Coupler Launch Conditions
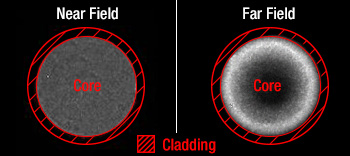
Figure 63B Near- and Far-Field Output Beam Profiles of Laser Launched into Multimode Fiber at Angle so that Cladding Modes Were Stripped.
Table 63C Tap Port Output Beam Profile | ||
---|---|---|
Coupling Ratio |
Near-Field Image | Far-Field Image |
50:50 | ![]() Click to Enlarge |
![]() Click to Enlarge |
75:25 | ![]() Click to Enlarge |
![]() Click to Enlarge |
90:10 | ![]() Click to Enlarge |
![]() Click to Enlarge |
99:1 | ![]() Click to Enlarge |
![]() Click to Enlarge |
Overview
Thorlabs' step-index multimode fiber couplers have characteristics that are mode dependent and therefore are affected by the launch condition used. In a waveguide, propagation modes are the electric field distributions that maintain an amplitude profile of constant shape in the transverse dimensions. Launch conditions describe how optical power is distributed within fiber in terms of spot size, NA, and/or mode fill. During manufacturing, Thorlabs ensures the performance of our step-index couplers by adhering to the launch condition standard of equilibrium modal distribution (EMD). EMD specifically constrains the propagation modes within step-index multimode fiber, as detailed in the EMD section below. For optimal performance, it is essential to achieve EMD when launching into our step-index multimode fiber couplers due to the various aspects of mode dependence inherent to the couplers. The mode dependence also causes the far-field beam profile of the tap port to be non-Gaussian, but this will not impact most applications (e.g. power monitoring).
Mode Dependence
Multimode (MM) fiber has several modal properties that distinguish it from single mode (SM) fiber. Compared to SM fiber, which only transmits one mode, MM fiber’s larger core size and higher NA provide the capacity for more guided modes. This facilitates a high coupling efficiency over a broad wavelength range and many transverse modes. However, the larger core and NA also mean a wide variety of launch conditions will have good transmission without necessarily fulfilling application-specific constraints such as uniform output beam profile.
For example, in the Multimode Fiber Beam Profiles Lab Fact, a laser was launched at 15° such that only skew rays propagated in the MM fiber. Skew rays are rays which never intersect the axis of the fiber, as shown in Figure 63A. This launch resulted in the beam profiles shown in Figure 63B, but still enabled >60% transmission compared to a 0° launch of meridional rays. Skew rays comprise higher-order guided modes. The higher the order of the mode, the less intensity will be concentrated in the center. The donut-shaped beam profile in the far field indicates that only higher-order guided modes are propagating. Although the donut looks similar to cladding mode propagation, cladding modes have been stripped in the setup for Figure 63B; this is evident in the absence of light in the cross-hatched cladding region. The use of a laser in this experiment provides a well-defined input modal distribution that allows the effects on the output beam profile to be clearly observed; however, many sources used with step-index MM couplers will not be collimated or coherent.
MM fiber with a step-index profile tends to maintain the same modal distribution (or mode fill) as the initial launch condition. Figure 63A shows how rays initially launched as skew rays will continue to propagate as skew rays. This property is in sharp contrast to another type of MM fiber, graded-index (GRIN), which continuously refocuses rays back towards the center. GRIN fibers will tend to output a Gaussian intensity distribution regardless of the input modal distribution. For step-index fibers, on the other hand, the preservation of input modal distribution makes the output beam profile heavily dependent on initial launch conditions.
Fused step-index fiber couplers exhibit an additional aspect of mode dependence, compared to step-index fiber by itself, due to the nature of evanescent wave coupling. Higher-order guided modes have a larger evanescent field, so they are preferentially coupled into the tap output before lower-order modes. Less light being coupled into the tap fiber will result in these higher-order modes dominating. For example, couplers with a coupling ratio of 99:1 will have predominantly lower-order mode output at the signal port and higher-order mode output at the tap port. In contrast, 50:50 couplers will have similar outputs from both the signal and tap ports. Due to the mode dependence of step-index fiber and multimode coupling, launch conditions for these couplers should closely match EMD, which is the standard used during manufacturing (see the EMD section below for more details).
Tap Port Non-Uniform Beam Profile
Even if an EMD launch is used, the preferential coupling of higher-order modes as described above will still cause a non-uniform beam profile in the tap port of couplers with coupling ratios other than 50:50. Higher-order modes, unlike the fundamental mode with its simple Gaussian profile, have complex profiles that may not concentrate intensity in the center of the core. Within the boundary conditions of the fiber, however, the combination of these higher-order modes will still be quite flat. Table 63C shows the near- and far-field profiles at the tap output ports of a multimode coupler using an EMD launch condition. The near-field images are excellent representations of the beam profile within the fiber, since there is as little free-space propagation as possible. As can be seen in the left column of images, even with a 99:1 coupling ratio, the beam profile of the tap port still appears flat within the fiber. The non-Gaussian effect of the higher-order guided modes only becomes evident in the far-field images shown in the right column.
For example, the 99:1 far-field image shows a clear donut profile. While this result seems to indicate cladding mode propagation, mode filtering was performed on the beam prior to it entering the coupler. The donut profile also becomes more pronounced with higher coupling ratios; 50:50 couplers will output flat profiles from both the signal and tap ports. Therefore, the far field donut profile of the 75:25, 90:10, and 99:1 coupling ratios is due to the preferential coupling of higher-order modes into the tap port. This behavior may affect applications where precise far-field intensity distribution in the tap port is needed. In those cases, a mode conditioner should be used on the output to achieve the desired output beam profile. Otherwise, this behavior should not affect most multimode coupler applications when these couplers are used with proper launch conditions.
EMD
Equilibrium modal distribution is a special condition for light propagating within a fiber where the modal distribution is independent of the length of fiber or initial mode fill conditions. In theory, any overfilled launch will trend toward EMD after propagating through an extremely long length of fiber (>300 m). In practicality, a radially flat intensity profile is a good indication that EMD has been achieved in a mode conditioning setup. For example, Figure 63D shows the cross-section line profile of the output from a 105 µm core fiber after mode conditioning. The profile is clearly quite flat across the core, which indicates that the mode conditioning was effective in achieving EMD.
A few steps are required to create launch conditions that satisfy EMD. First, an incoherent source must be used so that there are a large number of initial propagation modes. Then, an overfilled launch (see the Multimode Fiber Tutorial) is used to ensure all modes have at least some initial power in them. Without further mode conditioning, the attenuation of higher-order modes in the initial Gaussian intensity distribution will result in an altered beam profile further down the fiber; therefore, the next step is mode mixing (or mode scrambling), which can be done several ways. Methods include using a diffuser tip attached to the input of a patch cable or bending a patch cable in a serpentine, zigzag, or figure-eight configuration. After mode mixing, the beam profile should be fairly flat, but there could still be the possibility of cladding modes. The next step of mode filtering (or mode stripping) removes these unwanted cladding modes. A standard procedure for mode filtering is to tightly wrap the fiber five to ten times around a mandrel. Step-index MM couplers used with an incoherent source that is mode conditioned to achieve EMD standards will operate with optimal performance.
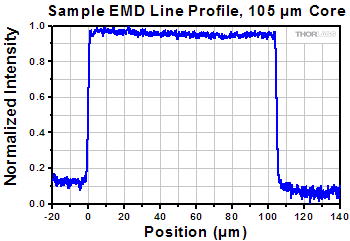
Click to Enlarge
Figure 63D Measured using a previous-generation M660F1 LED source coupled into an M18L01 patchcord, with a custom mode mixing and filtering setup.
Definition of 1x2 Fused Fiber Optic Coupler Specifications
This tab provides a brief explanation of how we determine several key specifications for our 1x2 couplers. 1x2 couplers are manufactured using the same process as our 2x2 fiber optic couplers, except the second input port is internally terminated using a proprietary method that minimizes back reflections. For combining light of different wavelengths, Thorlabs offers a line of single mode wavelength division multiplexers (WDMs). The ports on our 1x2 couplers are configured as shown in the schematic below.
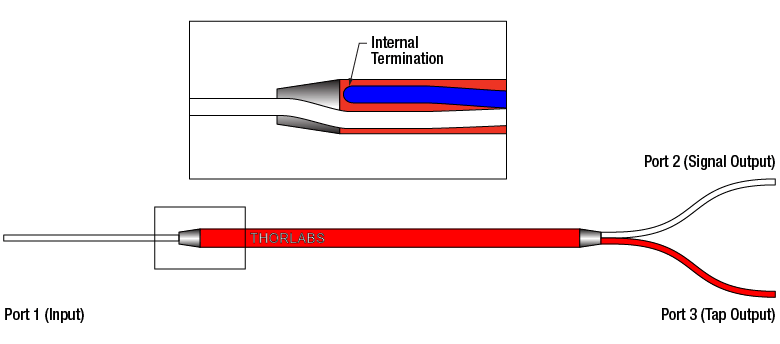
Excess Loss
Excess loss in dB is determined by the ratio of the total input power to the total output power:
Pport1 is the input power at port 1 and Pport2+Pport3 is the total output power from Ports 2 and 3. All powers are expressed in mW.
Polarization Dependent Loss (PDL)
The polarization dependent loss is defined as the ratio of the maximum and minimum transmissions due to polarization states in couplers. This specification pertains only to couplers not designed for maintaining polarization. PDL is always specified in decibels (dB), and can be calculated with the following equation:
where Pmax is the maximum power able to be transmitted through the coupler when scanning across all possible polarization states. Pmin is the minimum transmission across those same states.
Optical Return Loss (ORL) / Directivity
The directivity refers to the fraction of input light that is lost in the internally terminated fiber end within the coupler housing when port 1 is used as the input. It can be calculated in units of dB using the following equation:
where Pport1 and Pport1b are the optical powers (in mW) in port 1 and the internally terminated fiber, respectively. This output is the result of back reflection at the junction of the legs of the coupler and represents a loss in the total light output at ports 2 and 3. For a 50:50 coupler, the directivity is equal to the optical return loss (ORL).
Insertion Loss
The insertion loss is defined as the ratio of the input power to the output power at one of the output legs of the coupler (signal or tap). Insertion loss is always specified in decibels (dB). It is generally defined using the equation below:
where Pin and Pout are the input and output powers (in mW). For our 1x2 couplers, the insertion loss specification is provided for both signal and tap outputs; our specifications always list insertion loss for the signal output first. To define the insertion loss for a specific output (port 2 or port 3), the equation is rewritten as:
Insertion loss inherently includes both coupling (e.g., light transferred to the other output leg) and excess loss (e.g., light lost from the coupler) effects. The maximum allowed insertion loss for each output, signal and tap, are both specified. Because the insertion loss in each output is correlated to light coupled to the other output, no coupler will ever have the maximum insertion loss in both outputs simultaneously.
Calculating Insertion Loss using Power Expressed in dBm
Insertion loss can also be easily calculated with the power expressed in units of dBm. The equation below shows the relationship between power expressed in mW and dBm:
Then, the insertion loss in dB can be calculated as follows:
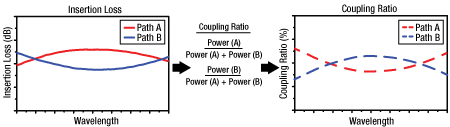
Click to Enlarge
A graphical representation of the coupling ratio calculation.
Coupling Ratio
Insertion loss (in dB) is the ratio of the input power to the output power from each leg of the coupler as a function of wavelength. It captures both the coupling ratio and the excess loss. The coupling ratio is calculated from the measured insertion loss. Coupling ratio (in %) is the ratio of the optical power from each output port (ports 2 and 3) to the sum of the total power of both output ports as a function of wavelength. Path A represents light traveling from port 1 to port 2 while Path B represents light traveling from port 1 to port 3. It is not impacted by spectral features such as the water absorption region because both output legs are affected equally.
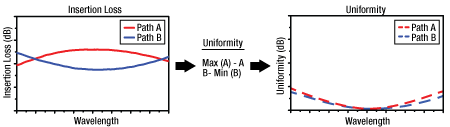
Click to Enlarge
A graphical representation of the Uniformity calculation.
Uniformity
The uniformity is also calculated from the measured insertion loss. Uniformity is the variation (in dB) of the insertion loss over the bandwidth. It is a measure of how evenly the insertion loss is distributed over the spectral range. The uniformity of Path A is the difference between the value of highest insertion loss and the solid red insertion loss curve (in the Insertion Plot above). The uniformity of Path B is the difference between the solid blue insertion loss curve and the value of lowest insertion loss.
Quick Links |
---|
Damage at the Air / Glass Interface |
Intrinsic Damage Threshold |
Preparation and Handling of Optical Fibers |
Laser-Induced Damage in Silica Optical Fibers
The following tutorial details damage mechanisms relevant to unterminated (bare) fiber, terminated optical fiber, and other fiber components from laser light sources. These mechanisms include damage that occurs at the air / glass interface (when free-space coupling or when using connectors) and in the optical fiber itself. A fiber component, such as a bare fiber, patch cable, or fused coupler, may have multiple potential avenues for damage (e.g., connectors, fiber end faces, and the device itself). The maximum power that a fiber can handle will always be limited by the lowest limit of any of these damage mechanisms.
While the damage threshold can be estimated using scaling relations and general rules, absolute damage thresholds in optical fibers are very application dependent and user specific. Users can use this guide to estimate a safe power level that minimizes the risk of damage. Following all appropriate preparation and handling guidelines, users should be able to operate a fiber component up to the specified maximum power level; if no maximum is specified for a component, users should abide by the "practical safe level" described below for safe operation of the component. Factors that can reduce power handling and cause damage to a fiber component include, but are not limited to, misalignment during fiber coupling, contamination of the fiber end face, or imperfections in the fiber itself. For further discussion about an optical fiber’s power handling abilities for a specific application, please contact Thorlabs’ Tech Support.
Damage at the Air / Glass Interface
There are several potential damage mechanisms that can occur at the air / glass interface. Light is incident on this interface when free-space coupling or when two fibers are mated using optical connectors. High-intensity light can damage the end face leading to reduced power handling and permanent damage to the fiber. For fibers terminated with optical connectors where the connectors are fixed to the fiber ends using epoxy, the heat generated by high-intensity light can burn the epoxy and leave residues on the fiber facet directly in the beam path.
Table 36C Estimated Optical Power Densities on Air / Glass Interfacea | ||
---|---|---|
Type | Theoretical Damage Thresholdb | Practical Safe Levelc |
CW (Average Power) |
~1 MW/cm2 | ~250 kW/cm2 |
10 ns Pulsed (Peak Power) |
~5 GW/cm2 | ~1 GW/cm2 |
Damage Mechanisms on the Bare Fiber End Face
Damage mechanisms on a fiber end face can be modeled similarly to bulk optics, and industry-standard damage thresholds for UV Fused Silica substrates can be applied to silica-based fiber. However, unlike bulk optics, the relevant surface areas and beam diameters involved at the air / glass interface of an optical fiber are very small, particularly for coupling into single mode (SM) fiber. therefore, for a given power density, the power incident on the fiber needs to be lower for a smaller beam diameter.
Table 36C lists two thresholds for optical power densities: a theoretical damage threshold and a "practical safe level". In general, the theoretical damage threshold represents the estimated maximum power density that can be incident on the fiber end face without risking damage with very good fiber end face and coupling conditions. The "practical safe level" power density represents minimal risk of fiber damage. Operating a fiber or component beyond the practical safe level is possible, but users must follow the appropriate handling instructions and verify performance at low powers prior to use.
Calculating the Effective Area for Single Mode Fibers
The effective area for single mode (SM) fiber is defined by the mode field diameter (MFD), which is the cross-sectional area through which light propagates in the fiber; this area includes the fiber core and also a portion of the cladding. To achieve good efficiency when coupling into a single mode fiber, the diameter of the input beam must match the MFD of the fiber.
As an example, SM400 single mode fiber has a mode field diameter (MFD) of ~Ø3 µm operating at 400 nm, while the MFD for SMF-28 Ultra single mode fiber operating at 1550 nm is Ø10.5 µm. The effective area for these fibers can be calculated as follows:
SM400 Fiber: Area = Pi x (MFD/2)2 = Pi x (1.5 µm)2 = 7.07 µm2 = 7.07 x 10-8 cm2
SMF-28 Ultra Fiber: Area = Pi x (MFD/2)2 = Pi x (5.25 µm)2 = 86.6 µm2 = 8.66 x 10-7 cm2
To estimate the power level that a fiber facet can handle, the power density is multiplied by the effective area. Please note that this calculation assumes a uniform intensity profile, but most laser beams exhibit a Gaussian-like shape within single mode fiber, resulting in a higher power density at the center of the beam compared to the edges. Therefore, these calculations will slightly overestimate the power corresponding to the damage threshold or the practical safe level. Using the estimated power densities assuming a CW light source, we can determine the corresponding power levels as:
SM400 Fiber: 7.07 x 10-8 cm2 x 1 MW/cm2 = 7.1 x 10-8 MW = 71 mW (Theoretical Damage Threshold)
7.07 x 10-8 cm2 x 250 kW/cm2 = 1.8 x 10-5 kW = 18 mW (Practical Safe Level)
SMF-28 Ultra Fiber: 8.66 x 10-7 cm2 x 1 MW/cm2 = 8.7 x 10-7 MW = 870 mW (Theoretical Damage Threshold)
8.66 x 10-7 cm2 x 250 kW/cm2 = 2.1 x 10-4 kW = 210 mW (Practical Safe Level)
Effective Area of Multimode Fibers
The effective area of a multimode (MM) fiber is defined by the core diameter, which is typically far larger than the MFD of an SM fiber. For optimal coupling, Thorlabs recommends focusing a beam to a spot roughly 70 - 80% of the core diameter. The larger effective area of MM fibers lowers the power density on the fiber end face, allowing higher optical powers (typically on the order of kilowatts) to be coupled into multimode fiber without damage.
Damage Mechanisms Related to Ferrule / Connector Termination
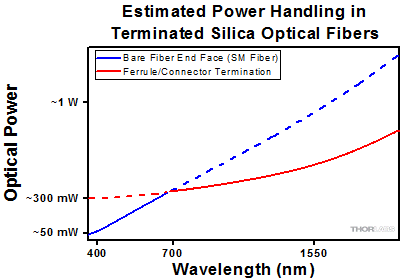
Figure 36D Plot showing approximate input power that can be incident on a single mode silica optical fiber with a termination. Each line shows the estimated power level due to a specific damage mechanism. The maximum power handling is limited by the lowest power level from all relevant damage mechanisms (indicated by a solid line).
Fibers terminated with optical connectors have additional power handling considerations. Fiber is typically terminated using epoxy to bond the fiber to a ceramic or steel ferrule. When light is coupled into the fiber through a connector, light that does not enter the core and propagate down the fiber is scattered into the outer layers of the fiber, into the ferrule, and the epoxy used to hold the fiber in the ferrule. If the light is intense enough, it can burn the epoxy, causing it to vaporize and deposit a residue on the face of the connector. This results in localized absorption sites on the fiber end face that reduce coupling efficiency and increase scattering, causing further damage.
For several reasons, epoxy-related damage is dependent on the wavelength. In general, light scatters more strongly at short wavelengths than at longer wavelengths. Misalignment when coupling is also more likely due to the small MFD of short-wavelength SM fiber that also produces more scattered light.
To minimize the risk of burning the epoxy, fiber connectors can be constructed to have an epoxy-free air gap between the optical fiber and ferrule near the fiber end face. Our high-power multimode fiber patch cables use connectors with this design feature.
Determining Power Handling with Multiple Damage Mechanisms
When fiber cables or components have multiple avenues for damage (e.g., fiber patch cables), the maximum power handling is always limited by the lowest damage threshold that is relevant to the fiber component. In general, this represents the highest input power that can be incident on the patch cable end face and not the coupled output power.
As an illustrative example, Figure 36D shows an estimate of the power handling limitations of a single mode fiber patch cable due to damage to the fiber end face and damage via an optical connector. The total input power handling of a terminated fiber at a given wavelength is limited by the lower of the two limitations at any given wavelength (indicated by the solid lines). A single mode fiber operating at around 488 nm is primarily limited by damage to the fiber end face (blue solid line), but fibers operating at 1550 nm are limited by damage to the optical connector (red solid line).
In the case of a multimode fiber, the effective mode area is defined by the core diameter, which is larger than the effective mode area for SM fiber. This results in a lower power density on the fiber end face and allows higher optical powers (on the order of kilowatts) to be coupled into the fiber without damage (not shown in graph). However, the damage limit of the ferrule / connector termination remains unchanged and as a result, the maximum power handling for a multimode fiber is limited by the ferrule and connector termination.
Please note that these are rough estimates of power levels where damage is very unlikely with proper handling and alignment procedures. It is worth noting that optical fibers are frequently used at power levels above those described here. However, these applications typically require expert users and testing at lower powers first to minimize risk of damage. Even still, optical fiber components should be considered a consumable lab supply if used at high power levels.
Intrinsic Damage Threshold
In addition to damage mechanisms at the air / glass interface, optical fibers also display power handling limitations due to damage mechanisms within the optical fiber itself. These limitations will affect all fiber components as they are intrinsic to the fiber itself. Two categories of damage within the fiber are damage from bend losses and damage from photodarkening.
Bend Losses
Bend losses occur when a fiber is bent to a point where light traveling in the core is incident on the core/cladding interface at an angle higher than the critical angle, making total internal reflection impossible. Under these circumstances, light escapes the fiber, often in a localized area. The light escaping the fiber typically has a high power density, which burns the fiber coating as well as any surrounding furcation tubing.
A special category of optical fiber, called double-clad fiber, can reduce the risk of bend-loss damage by allowing the fiber’s cladding (2nd layer) to also function as a waveguide in addition to the core. By making the critical angle of the cladding/coating interface higher than the critical angle of the core/clad interface, light that escapes the core is loosely confined within the cladding. It will then leak out over a distance of centimeters or meters instead of at one localized spot within the fiber, minimizing the risk of damage. Thorlabs manufactures and sells 0.22 NA double-clad multimode fiber, which boasts very high, megawatt range power handling.
Photodarkening
A second damage mechanism, called photodarkening or solarization, can occur in fibers used with ultraviolet or short-wavelength visible light, particularly those with germanium-doped cores. Fibers used at these wavelengths will experience increased attenuation over time. The mechanism that causes photodarkening is largely unknown, but several fiber designs have been developed to mitigate it. For example, fibers with a very low hydroxyl ion (OH) content have been found to resist photodarkening and using other dopants, such as fluorine, can also reduce photodarkening.
Even with the above strategies in place, all fibers eventually experience photodarkening when used with UV or short-wavelength light, and thus, fibers used at these wavelengths should be considered consumables.
Preparation and Handling of Optical Fibers
General Cleaning and Operation Guidelines
These general cleaning and operation guidelines are recommended for all fiber optic products. Users should still follow specific guidelines for an individual product as outlined in the support documentation or manual. Damage threshold calculations only apply when all appropriate cleaning and handling procedures are followed.
-
All light sources should be turned off prior to installing or integrating optical fibers (terminated or bare). This ensures that focused beams of light are not incident on fragile parts of the connector or fiber, which can possibly cause damage.
-
The power-handling capability of an optical fiber is directly linked to the quality of the fiber/connector end face. Always inspect the fiber end prior to connecting the fiber to an optical system. The fiber end face should be clean and clear of dirt and other contaminants that can cause scattering of coupled light. Bare fiber should be cleaved prior to use and users should inspect the fiber end to ensure a good quality cleave is achieved.
-
If an optical fiber is to be spliced into the optical system, users should first verify that the splice is of good quality at a low optical power prior to high-power use. Poor splice quality may increase light scattering at the splice interface, which can be a source of fiber damage.
-
Users should use low power when aligning the system and optimizing coupling; this minimizes exposure of other parts of the fiber (other than the core) to light. Damage from scattered light can occur if a high power beam is focused on the cladding, coating, or connector.
Tips for Using Fiber at Higher Optical Power
Optical fibers and fiber components should generally be operated within safe power level limits, but under ideal conditions (very good optical alignment and very clean optical end faces), the power handling of a fiber component may be increased. Users must verify the performance and stability of a fiber component within their system prior to increasing input or output power and follow all necessary safety and operation instructions. The tips below are useful suggestions when considering increasing optical power in an optical fiber or component.
-
Splicing a fiber component into a system using a fiber splicer can increase power handling as it minimizes possibility of air/fiber interface damage. Users should follow all appropriate guidelines to prepare and make a high-quality fiber splice. Poor splices can lead to scattering or regions of highly localized heat at the splice interface that can damage the fiber.
-
After connecting the fiber or component, the system should be tested and aligned using a light source at low power. The system power can be ramped up slowly to the desired output power while periodically verifying all components are properly aligned and that coupling efficiency is not changing with respect to optical launch power.
-
Bend losses that result from sharply bending a fiber can cause light to leak from the fiber in the stressed area. When operating at high power, the localized heating that can occur when a large amount of light escapes a small localized area (the stressed region) can damage the fiber. Avoid disturbing or accidently bending fibers during operation to minimize bend losses.
-
Users should always choose the appropriate optical fiber for a given application. For example, large-mode-area fibers are a good alternative to standard single mode fibers in high-power applications as they provide good beam quality with a larger MFD, decreasing the power density on the air/fiber interface.
-
Step-index silica single mode fibers are normally not used for ultraviolet light or high-peak-power pulsed applications due to the high spatial power densities associated with these applications.
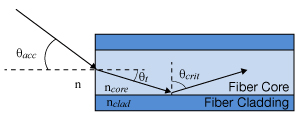
Click to Enlarge
Figure 79A Total Internal Reflection in an Optical Fiber
Guiding Light in an Optical Fiber
Optical fibers are part of a broader class of optical components known as waveguides that utilize total internal reflection (TIR) in order to confine and guide light within a solid or liquid structure. Optical fibers, in particular, are used in numerous applications; common examples include telecommunications, spectroscopy, illumination, and sensors.
One of the more common glass (silica) optical fibers uses a structure known as a step-index fiber, which is shown in Figure 79A. Step-index fibers have an inner core made from a material with a refractive index that is higher than the surrounding cladding layer. Within the fiber, a critical angle of incidence exists such that light will reflect off the core/cladding interface rather than refract into the surrounding medium. To fulfill the conditions for TIR in the fiber, the angle of incidence of light launched into the fiber must be less than a certain angle, which is defined as the acceptance angle, θacc. Snell's law can be used to calculate this angle:
where ncore is the refractive index of the fiber core, nclad is the refractive index of the fiber cladding, n is the refractive index of the outside medium, θcrit is the critical angle, and θacc is the acceptance half-angle of the fiber. The numerical aperture (NA) is a dimensionless quantity used by fiber manufacturers to specify the acceptance angle of an optical fiber and is defined as:
In step-index fibers with a large core (multimode), the NA can be calculated directly using this equation. The NA can also be determined experimentally by tracing the far-field beam profile and measuring the angle between the center of the beam and the point at which the beam intensity is 5% of the maximum; however, calculating the NA directly provides the most accurate value.
Number of Modes in an Optical Fiber
Each potential path that light propagates through in an optical fiber is known as a guided mode of the fiber. Depending on the physical dimensions of the core/cladding regions, refractive index, and wavelength, anything from one to thousands of modes can be supported within a single optical fiber. The two most commonly manufactured variants are single mode fiber (which supports a single guided mode) and multimode fiber (which supports a large number of guided modes). In a multimode fiber, lower-order modes tend to confine light spatially in the core of the fiber; higher-order modes, on the other hand, tend to confine light spatially near the core/cladding interface.
Using a few simple calculations, it is possible to estimate the number of modes (single mode or multimode) supported by an optical fiber. The normalized optical frequency, also known as the V-number, is a dimensionless quantity that is proportional to the free space optical frequency but is normalized to guiding properties of an optical fiber. The V-number is defined as:
where V is the normalized frequency (V-number), a is the fiber core radius, and λ is the free space wavelength. Multimode fibers have very large V-numbers; for example, a Ø50 µm core, 0.39 NA multimode fiber at a wavelength of 1.5 µm has a V-number of 40.8.
For multimode fiber, which has a large V-number, the number of modes supported is approximated using the following relationship.
In the example above of the Ø50 µm core, 0.39 NA multimode fiber, it supports approximately 832 different guided modes that can all travel simultaneously through the fiber.
Single mode fibers are defined with a V-number cut-off of V < 2.405, which represents the point at which light is coupled only into the fiber's fundamental mode. To meet this condition, a single mode fiber has a much smaller core size and NA compared to a multimode fiber at the same wavelength. One example of this, SMF-28 Ultra single mode fiber, has a nominal NA of 0.14 and an Ø8.2 µm core at 1550 nm, which results in a V-number of 2.404.
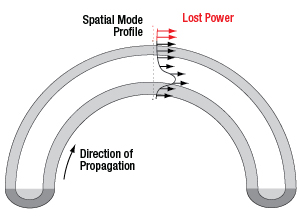
Click to Enlarge
Figure 79B Attenuation Due to Macrobend Loss
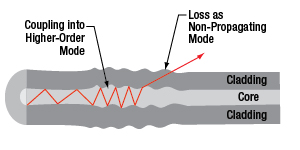
Click to Enlarge
Figure 79C Attenuation Due to Microbend Loss
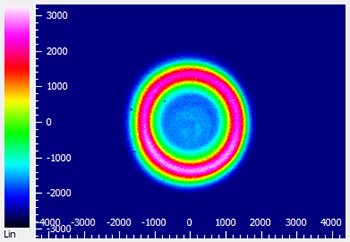
Click to Enlarge
Figure 79D Beam profile measurement of FT200EMT multimode fiber and a former generation M565F1 LED (replaced by the M565F3) showing light guided in the cladding rather than the core of the fiber.
Sources of Attenuation
Loss within an optical fiber, also referred to as attenuation, is characterized and quantified in order to predict the total transmitted power lost within a fiber optic setup. The sources of these losses are typically wavelength dependent and range from the material used in the fiber itself to bending of the fiber. Common sources of attenuation are detailed below:
Absorption
Because light in a standard optical fiber is guided via a solid material, there are losses due to absorption as light propagates through the fiber. Standard fibers are manufactured using fused silica and are optimized for transmission from 1300 nm to 1550 nm. At longer wavelengths (>2000 nm), multi-phonon interactions in fused silica cause significant absorption. Fluoride glasses such as ZrF4 and InF3 are used in manufacturing Mid-IR optical fibers primarily because they exhibit lower loss at these wavelengths. ZrF4 and InF3 fibers have a multi-phonon edge of ~3.6 µm and ~4.6 µm, respectively.
Contaminants in the fiber also contribute to the absorption loss. One example of an undesired impurity is water molecules that are trapped in the glass of the optical fiber, which will absorb light around 1300 nm and 2.94 µm. Since telecom signals and some lasers operate in that same region, any water molecules present in the fiber will attenuate the signal significantly.
The concentration of ions in the fiber glass is often controlled by manufacturers to tune the transmission/attenuation properties of a fiber. For example, hydroxyl ions (OH-) are naturally present in silica and absorb light in the NIR-IR spectrum. Therefore, fibers with low-OH content are preferred for transmission at telecom wavelengths. On the other hand, fibers with high-OH content typically exhibit increased transmission at UV wavelengths and thus may be preferred by users interested in applications such as fluorescence or UV-VIS spectroscopy.
Scattering
For the majority of fiber optics applications, light scattering is a source of loss that occurs when light encounters a change in the refractive index of the medium. These changes can be extrinsic, caused by impurities, particulates, or bubbles; or intrinsic, caused by fluctuations in the glass density, composition, or phase state. Scattering is inversely related to the wavelength of light, so scattering loss becomes significant at shorter wavelengths such as the UV or blue regions of the spectrum. Using proper fiber cleaning, handling, and storage procedures may minimize the presence of impurities on tips of fibers that cause large scattering losses.
Bending Loss
Losses that occur due to changes in the external and internal geometry of an optical fiber are known as bending loss. These are usually separated into two categories: macrobending loss and microbending loss.
Macrobend loss is typically associated with the physical bending of an optical fiber; for example, rolling it in a tight coil. As shown in Figure 79D, guided light is spatially distributed within the core and cladding regions of the fiber. When a fiber is bent at a radius, light near the outer radius of the bend cannot maintain the same spatial mode profile without exceeding the speed of light. Instead, the energy is lost to the surroundings as radiation. For a large bend radius, the losses associated with bending are small; however, at bend radii smaller than the recommended bend radius of a fiber, bend losses become very significant. For short periods of time, optical fibers can be operated at a small bend radius; however, for long-term storage, the bend radius should be larger than the recommended value. Use proper storage conditions (temperature and bend radius) to reduce the likelihood of permanently damaging the fiber; the FSR1 Fiber Storage Reel is designed to minimize high bend loss.
Microbend loss arises from changes in the internal geometry of the fiber, particularly the core and cladding layers. These random variations (i.e., bumps) in the fiber structure disturb the conditions needed for total internal reflection, causing propagating light to couple into a non-propagating mode that leaks from the fiber (see Figure 79D for details). Unlike macrobend loss, which is controlled by the bend radius, microbend loss occurs due to permanent defects in the fiber that are created during fiber manufacturing.
Cladding Modes
While most light in a multimode fiber is guided via TIR within the core of the fiber, higher-order modes that guide light within both the core and cladding layer, because of TIR at the cladding and coating/buffer interface, can also exist. This results in what is known as a cladding mode. An example of this can be seen in the beam profile measurement in Figure 79D, which shows cladding modes with a higher intensity in the cladding than in the core of the fiber. These modes can be non-propagating (i.e., they do not fulfill the conditions for TIR) or they can propagate over a significant length of fiber. Because cladding modes are typically higher-order, they are a source of loss in the presence of fiber bending and microbending defects. Cladding modes are also lost when connecting two fibers via connectors as they cannot be easily coupled between optical fibers.
Cladding modes may be undesired for some applications (e.g., launching into free space) because of their effect on the beam spatial profile. Over long fiber lengths, these modes will naturally attenuate. For short fiber lengths (<10 m), one method for removing cladding modes from a fiber is to use a mandrel wrap at a radius that removes cladding modes while keeping the desired propagating modes.
Launch Conditions
Underfilled Launch Condition
For a large multimode fiber which accepts light over a wide NA, the condition of the light (e.g., source type, beam diameter, NA) coupled into the fiber can have a significant effect on performance. An underfilled launch condition occurs when the beam diameter and NA of light at the coupling interface are smaller than the core diameter and NA of the fiber. A common example of this is launching a laser source into a large multimode fiber. As seen in Figure 79E, underfilled launches tend to concentrate light spatially in the center of the fiber, filling lower-order modes preferentially over higher-order modes. As a result, they are less sensitive to macrobend losses and do not have cladding modes. The measured insertion loss for an underfilled launch tends to be lower than typical, with a higher power density in the core of the fiber.
Overfilled Launch Condition
Overfilled launches are defined by situations where the beam diameter and NA at the coupling interface are larger than the core diameter and NA of the fiber. One method to achieve this is by launching light from an LED source into a small multimode fiber. An overfilled launch completely exposes the fiber core and some of the cladding to light, enabling the filling of lower- and higher-order modes equally (as seen in Figure 79F) and increasing the likelihood of coupling into cladding modes of the fiber. This increased percentage of higher-order modes means that overfilled fibers are more sensitive to bending loss. The measured insertion loss for an overfilled launch tends to be higher than typical, but results in an overall higher output power compared to an underfilled fiber launch.
There are advantages and disadvantages to underfilled or overfilled launch conditions, depending on the needs of the intended application. For measuring the baseline performance of a multimode fiber, Thorlabs recommends using a launch condition where the beam diameter is 70-80% of the fiber core diameter. Over short distances, an overfilled fiber has more output power; however, over long distances (>10 - 20 m) the higher-order modes that more susceptible to attenuation will disappear.
Posted Comments: | |
dave Hofeldt
 (posted 2021-09-21 13:42:38.437) I cannot find the length of any of the "arms" on the 1x2 couplers YLohia
 (posted 2021-09-21 02:12:26.0) Hello, the length of each arm is specified in the engineering drawing, as well as the on specsheet ("Fiber Lead Length and Tolerance"), as 0.8 m +0.075 m / -0.0 m. This spec is true for the input (common) arm as well as the two output arms. Francesco Lena
 (posted 2019-09-18 13:38:38.287) Hello! Is it possible to ship the TT200SS1A Coupler with different cable lengths?
Thank you very much YLohia
 (posted 2019-09-19 08:38:40.0) Hello Francesco, thank you for contacting Thorlabs. We will reach out to you directly to discuss the possibility of offering these custom couplers. |

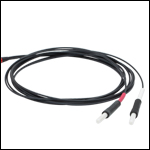
Click for Details
Couplers with ceramic ferrules on the output ports have black tubing.

Click for Details
Couplers with connectors on the output ports have white and red tubing.
- 1x2 Multimode Couplers with 50:50 Split Ratio
- 400 - 900 nm or 400 - 2200 nm Wavelength Range
- Available with SMA905 or FC/PC Connectors
- Select Items Feature Output Ports with Ø1.25 mm or Ø2.5 mm Ceramic Ferrules
These 50:50 split ratio couplers feature Ø200 µm core, 0.39 NA fiber with a 400 - 900 nm (high OH) or 400 - 2200 nm (low OH) wavelength range. All couplers have a jacket of Ø900 µm Hytrel®* loose tubing.
Couplers are offered with FC/PC or SMA905 connectors on all ports. Alternatively, we offer couplers with SMA905 or FC/PC connectors on the input port and either Ø1.25 mm or Ø2.5 mm ceramic ferrules on the output ports. These couplers have black tubing on all ports to minimize light leakage with white or red heat-shrink wrap to indicate different ports. The couplers highlighted green in the table below are commonly used in optogenetics applications.
*Hytrel® is a registered trademark of DuPont Polymers, Inc.
Item # | Info | Wavelength Range |
Coupling Ratioa,b |
Coupling Ratio Tolerancea |
Insertion Lossa,b | Excess Lossa,b |
Max Power Levelc | Core / Cladding Diameter |
Fiberd | Termination |
---|---|---|---|---|---|---|---|---|---|---|
TT200R5S1A | ![]() |
400 - 900 nm (High OH) |
50:50 | ±5.0% | ≤4.2 dB / ≤4.2 dB | <0.65 dB (Typ.) |
5 W (Connectors or Bare); 10 W (Spliced) |
200 µm / 225 µm | FT200UMT MM Fiber, 0.39 NA |
SMA905 |
TT200R5F1A | ![]() |
FC/PC | ||||||||
TT200R5S1B | ![]() |
400 - 2200 nm (Low OH) |
FT200EMT MM Fiber, 0.39 NA |
SMA905 | ||||||
TT200R5F1B | ![]() |
FC/PC | ||||||||
TT200SL1A | ![]() |
400 - 900 nm (High OH) |
50:50 | ±5.0% | ≤4.2 dB / ≤4.2 dB | <0.65 dB (Typ.) |
5 W (Connectors or Bare); 10 W (Spliced) |
200 µm / 225 µm | FT200UMT MM Fiber, 0.39 NA |
SMA905 to Ø1.25 mm Ferrules |
TT200FL1A | ![]() |
FC/PC to Ø1.25 mm Ferrules |
||||||||
TT200SS1A | ![]() |
SMA905 to Ø2.5 mm Ferrules |
||||||||
TT200FS1A | ![]() |
FC/PC to Ø2.5 mm Ferrules |

Item # | Info | Wavelength Range |
Coupling Ratioa,b |
Coupling Ratio Tolerancea |
Insertion Lossa,b | Excess Lossa,b |
Max Power Levelc | Core / Cladding Diameter |
Fiberd | Termination |
---|---|---|---|---|---|---|---|---|---|---|
TT200R3S1A | ![]() |
400 - 900 nm (High OH) |
75:25 | ±3.0% | ≤2.0 dB / ≤7.2 dB | <0.5 dB (Typ.) |
5 W (Connectors or Bare); 10 W (Spliced) |
200 µm / 225 µm | FT200UMT MM Fiber, 0.39 NA |
SMA905 |
TT200R3F1A | ![]() |
FC/PC | ||||||||
TT200R3S1B | ![]() |
400 - 2200 nm (Low OH) |
FT200EMT MM Fiber, 0.39 NA |
SMA905 | ||||||
TT200R3F1B | ![]() |
FC/PC |

Item # | Info | Wavelength Range |
Coupling Ratioa,b |
Coupling Ratio Tolerancea |
Insertion Lossa,b | Excess Lossa,b |
Max Power Levelc | Core / Cladding Diameter |
Fiberd | Termination |
---|---|---|---|---|---|---|---|---|---|---|
TT200R2S1A | ![]() |
400 - 900 nm (High OH) |
90:10 | ±1.5% | ≤1.1 dB / ≤11.3 dB | <0.5 dB (Typ.) |
5 W (Connectors or Bare); 10 W (Spliced) |
200 µm / 225 µm | FT200UMT MM Fiber, 0.39 NA |
SMA905 |
TT200R2F1A | ![]() |
FC/PC | ||||||||
TT200R2S1B | ![]() |
400 - 2200 nm (Low OH) |
FT200EMT MM Fiber, 0.39 NA |
SMA905 | ||||||
TT200R2F1B | ![]() |
FC/PC |